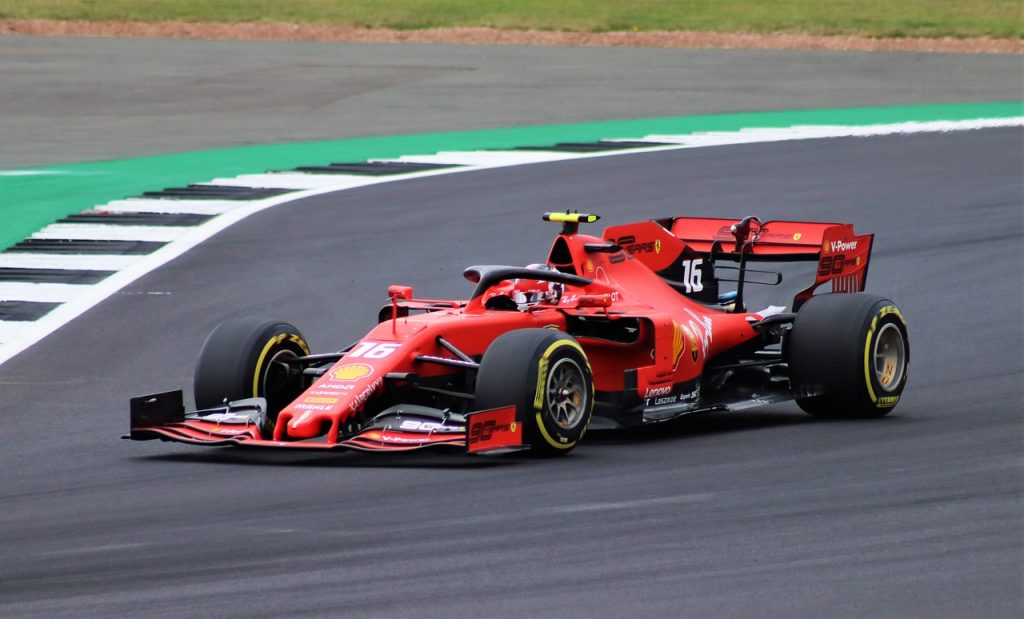
Introduction
In automotive and motorsport engineering, optimal performance depends on a lot of factors such as aerodynamics, engine specifications and vehicle dynamics plays a key role too. Vehicle dynamics, for those who are new to the field, refers to how a vehicle moves and responds in different manoeuvres such as cornering, braking, and accelerating. This is why it is an important topic to understand for superior handling and performance both on the road and on track. The key aspects of vehicle dynamics are Tyre Dynamics, Longitudinal Dynamics, Lateral Dynamics and Vertical Dynamics, each of which will be examined in this blog post.
Tyre Dynamics
Tyres are important for performance and handling because they are the only part of the car to be in contact with the ground (this connection is known as the contact patch).
Any forces that tyres produce act at the contact patch. One such force is the lateral force (responsible for vehicle cornering), which we see at the rear of the contact patch. The distance from the center of the contact patch to the point where the lateral force is applied is known as the pneumatic trail. During cornering, a slip angle is created and as a result lateral force is generated. Slip angle is the angle between the direction in which a wheel is pointing and the direction in which it is actually travelling.
Tyres are also the source of traction (grip), braking and cornering forces. To have maximum grip the contact patch area needs to be maximised which is why most high performance tyres are wider standard tyres.
The ‘slick’ tyres used in F1 have no treads and this means that they can have high levels of grip. Slick tyres are softer, have faster wear and also need higher temperatures to be able to provide these levels of grip. In wet conditions, using slicks can cause hydroplaning (when tyres lose grip with the road and instead travel on a film of water sitting on top of the road) so wet tyres have treads to displace water for improved grip. In regular road cars, it is impractical to keep changing tyres depending on the weather so using tyres with treads is the common standard. We can see a further illustration of the relationship between grip and traction by the use of snow tyres or chains in places where driving in icy weather is more common (though not in F1 as of yet!).
It is possible to simulate tyre behaviour and there are multiple tyre modelling methods, but the most widely used in motorsport and automotive is the Pacejka Tyre model. It is also known as the magic formula because it is a semi-empirical model (this will be covered in a future blog).
Want to know more about tyres and the role of a tyre engineer? Check out Vehicle Dynamics in F1 HERE!
Longitudinal Dynamics
This covers the straight-line performance of a car (accelerating and braking). It considers effects such as aerodynamics (drag and downforce), car setup, tyre toe and camber etc. It is important to account for the weight transfer and aerodynamic loads between axles because the car dives (front axle vertical load increases and rear axle vertical load decreases) during braking and squats (front axle vertical load decreases and rear axle vertical load increases) during traction (acceleration). It is useful to quantify the total downforce, total drag, ride height, balance, and camber.
Lateral Dynamics
The simplest model to examine lateral dynamics is the bicycle model because it provides a good understanding of vehicle behaviour but some more complex models, such as MBS, are used in vehicle development. It is a vehicle model with 2DoF: sideslip and yaw rate as a response to steering input.
The bicycle model makes the following assumptions:
- Considering just a single wheel and you are lumping in there both forces
- Longitudinal speed is assumed to be constant.
- No longitudinal weight transfer
- No roll or suspension movement
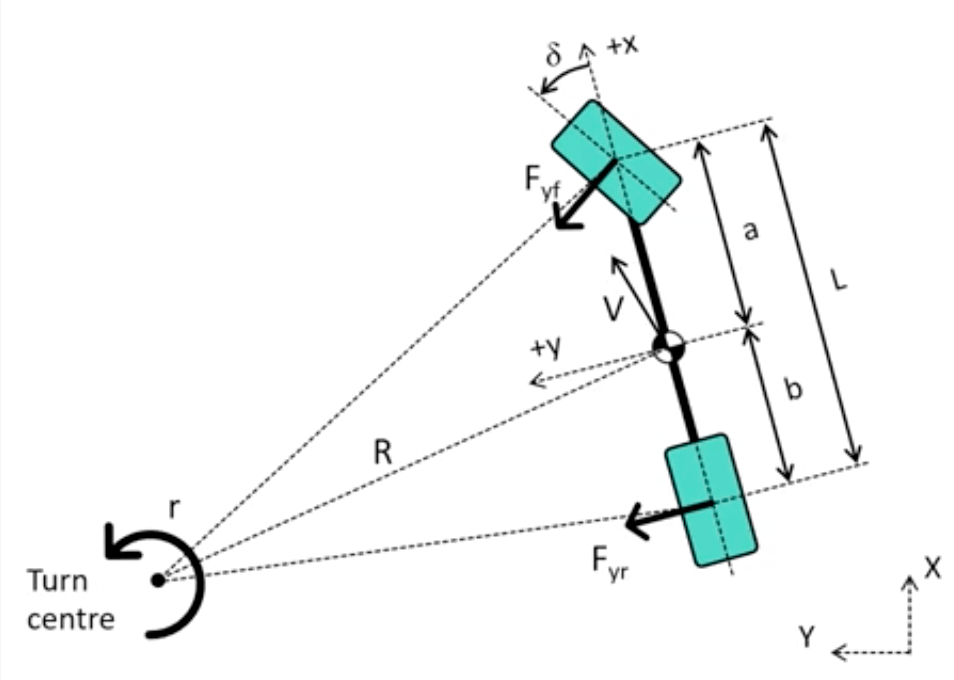
From this model, different steering geometries can be examined. In automotive engineering, Ackermann steering geometry (which involves turning the inner wheel more than the outer wheel for smoother turns) is widely used because the aim is to avoid excessive tyre wear as much as possible, especially during low speed cornering.
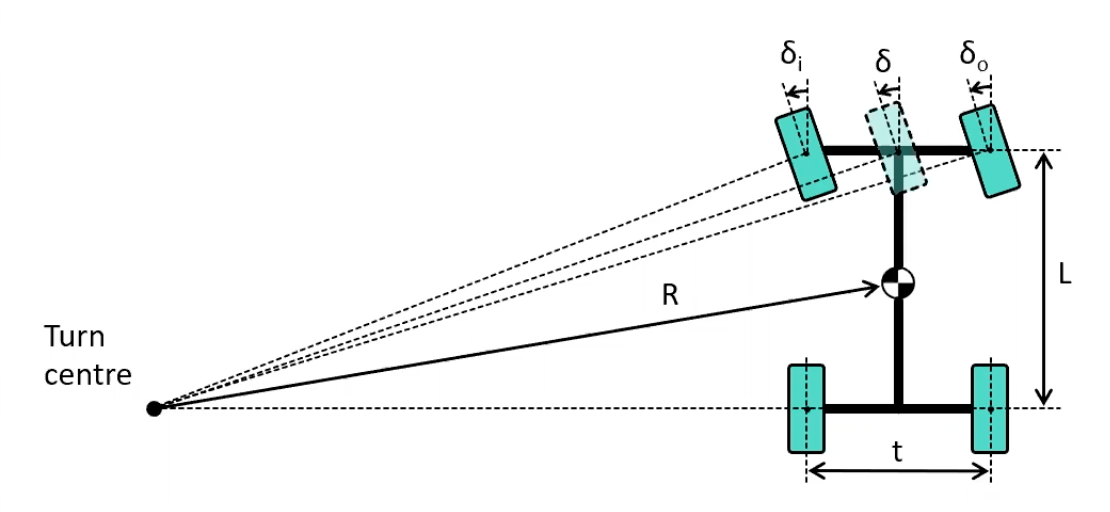
In Formula 1, anti-Ackermann geometry is used (the outer wheel turns more than the inner wheel) because this brings higher slip angles in the outer wheel which has more load. This generates more force and can corner more (the higher the sideslip, the higher the tyre force, and the outer wheel has a higher normal force thus higher cornering power). One drawback to this is that during low-speed cornering, the wheel drag and tyre wear are incredibly high.
During high-speed cornering, understeer is when you lose grip at the front first and hit a wall with the front of the car during a crash while oversteer is when you lose grip at the rear and hit a wall with the rear of the car. These understeer/oversteer characteristics can also be derived from the bicycle model.
Ideally, the understeer gradient should be 0 to have a neutral steer system because neutral steering cars are close to being critically damped, which corresponds to the fastest cornering response that a vehicle can have. But in reality, vehicle dynamicists aim to get as close to neutral steer but in understeer (positive understeer gradient) as possible.
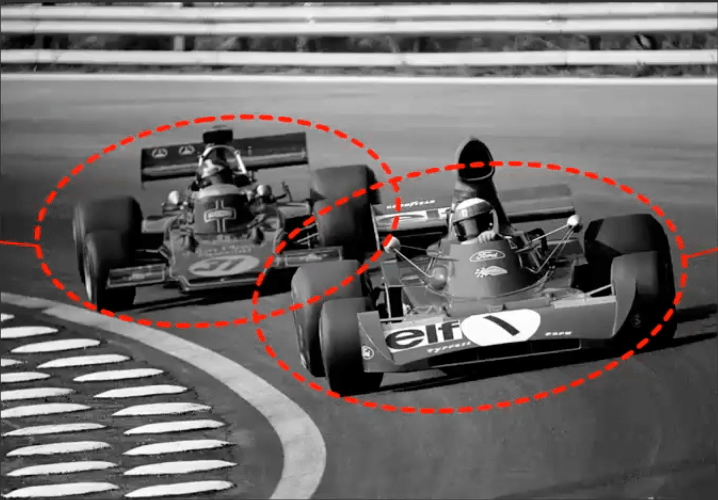
Vertical Dynamics
The goal when studying vertical dynamics is to find a balance between comfort (isolating the vehicle from the ground) and performance (minimizing the tyre normal force variation) that depends on the vehicle application.
For highly aerodynamic vehicles, the focus is on keeping the optimum vehicle ride height and attitude to get the most out of the aerodynamics package. In motorsport, the focus is on performance, and in competitions with highly aerodynamic packages (like F1), the focus is on aerodynamics.
In automotive, both comfort and performance are focused on providing the drivers with a mix of the two because the distances that are to be covered by automotive cars over the life cycle are significant.
It can be evaluated using a 2DoF Quarter car model, a 4DoF (half car model), 7DoF or 14DoF models.
The simplest model to understand for initial target setting is the 2 DoF quarter car model because it accounts for the sprung mass (the body) and the unsprung mass (the tyres, wheels and part of the suspension). Solving the equations of the quarter car model can provide information on the type of response the suspension will provide to certain scenarios. Similar to lateral dynamics, the damping ratio should ideally be underdamped to focus on comfort and performance. If the focus is on aerodynamics (such as in F1 cars) the damping ratios would be higher but should not reach 1.
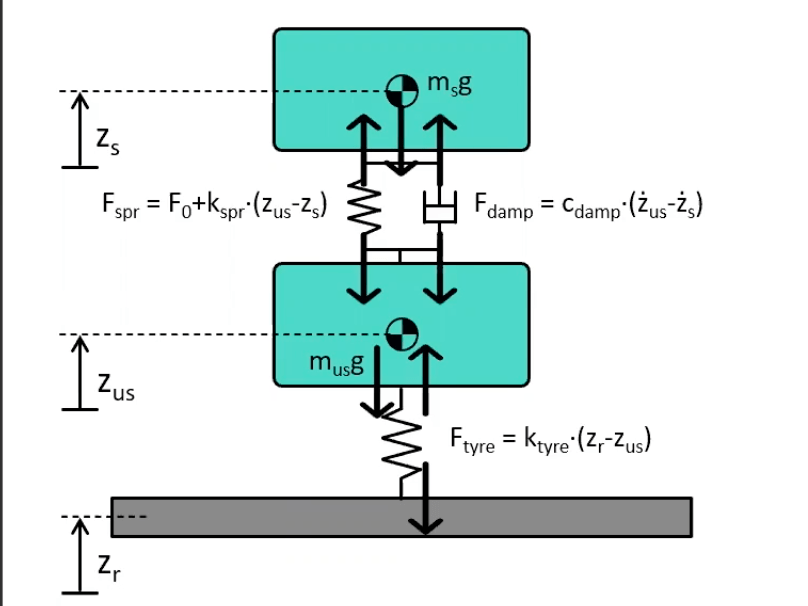
Vehicle Dynamics in F1 course leader Marc Ollé Bernades and Vehicle Dynamics Modelling and Simulation course leader Javier Sistiaga Vidal-Ribas comprehensively cover all these topics in more detail and use case studies for clearer understanding.
Structure of Vehicle Dynamics in Industry
In the automotive industry, vehicle dynamics aim to deliver optimal performance, safety and comfort. There is usually a balance between performance and comfort because the most comfortable setup does not provide the most performance and vice versa.
In more affordable cars, comfort is prioritised, while in high-performance vehicles such as the ones used in motorsport performance is prioritised. In motorsport performance over comfort is preferred because the focus is on speed, handling, and the competitive edge.
In both industries, vehicle dynamicists can work in some of the following departments:
- Tyres – focus on understanding tyre behaviour and characteristics such as grip, tread design, and compound composition.
- Suspension (Ride) – responsible for designing and tuning suspension systems
- In the automotive industry, to achieve a balance between ride comfort and handling performance
- In the motorsport industry, to extract maximum performance
- Driver in the Loop – focuses on human-machine interaction aspects, considering how driver feedback with the car during different driving scenarios
- Modelling and Simulation (will be covered in more detail in a future blog post) – create a computational model of the car to simulate behaviour in different conditions.
In motorsport, the roles of race engineer and performance engineer require a deep understanding of these areas to optimise the ride setup for drivers on any particular track.
Conclusion
By understanding and optimizing these principles, engineers have been pushing the boundaries of automotive and motorsport ride and handling, delivering vehicles that offer a perfect balance of agility, stability, and comfort.
As technology advances and integration with active systems continues, the quest for superior vehicle dynamics continues, promising ever-evolving innovations and enhancements for enthusiasts and drivers around the world.
Be sure to look out for the next blog, where the modelling and simulation processes for some of these principles will be explained further.
Meet the Author
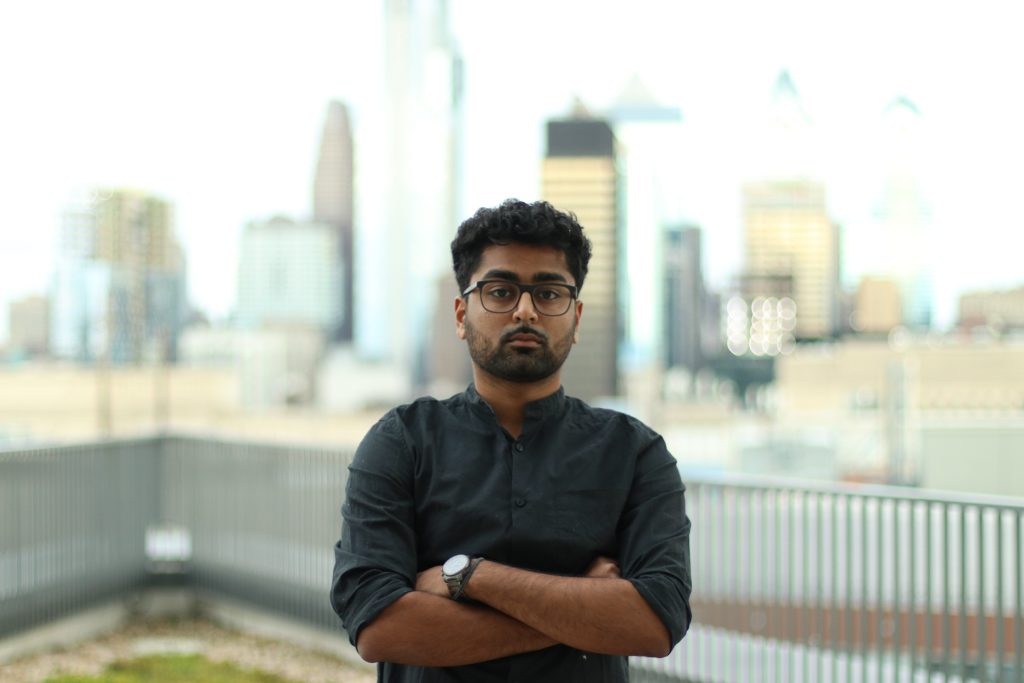
Dheer is currently pursuing an MSc in Automotive Engineering at Cranfield University after completing his Bachelor’s in Mechanical Engineering in the US. He has built his experience through not only his studies but also by investing in his passion for h igh-performance vehicles through courses and hands-on opportunities. Outside engineering, he enjoys following F1 and other sports (like golf and football to name a few).